Stay Up to Date
Submit your email address to receive the latest industry and Aerospace America news.
One hurdle has been the inability to accurately model partially melted ice crystals
In a freezer room on the campus of Penn State University, a partially melted ice crystal levitates motionless on acoustic waves. Green laser light bathes the crystal to distinguish the liquid exterior from its icy core. On command, an air gun pushes a stainless steel rod toward the crystal at 100 meters per second down a barrel that stops the rod at the moment of impact. The result? The crystal shatters, and specks of ice fuse to the tip of the rod, just as they would if the crystal smacked into a surface inside a jet engine. A high-speed camera records everything for the research team.
This method of capturing a host of characteristics about partially melted ice crystals, from their ice content to how they fracture and splash, was described to me by Jose Palacios, assistant professor of aerospace engineering and head of the aircraft icing research team at Penn State. The team plans to continue gathering data this way through September.
The technique is emerging as a possible linchpin in U.S. efforts to solve the rare but potentially fatal problem of high-altitude engine icing. That happens when a jet cruising in the seemingly clear air around major thunderstorms ingests a high volume of a particular kind of ice crystal that’s prone to partially melting and refreezing. The plane’s radar can’t see these crystals, and the pilot may not immediately notice them bouncing off the exterior like grains of sand no more than 1 millimeter across.
Inside the jet’s engines, though, warm air partially melts the crystals before they strike the surfaces of the compressor located before the combuster. The sheer volume of these partially melted crystals cools these surfaces below freezing, causing the drops to accumulate, or accrete, in the form of ice. Chunks as large as a few pounds can build up and break off and snuff combustion or damage the engine. Since 2015, the phenomena has caused a combined 162 cases of either complete engine flameout or stalling, a temporary loss of power due to restricted airflow, and damaged compressors, according to data gathered by Boeing. So far, no crashes have resulted. Right now, pilots cope by flying around huge swaths of weather that might or might not lead to icing. Engine stalls typically last less than a few seconds, but when flameouts occur, as a last resort pilots can descend to 10,000 feet where the ice melts and the pilots can restart their engine or engines.
Researchers want to solve the icing problem before luck runs out. Engineers have over the years made minor modifications to in-service engines to cope with icing. In 2015 GE ordered modifications to some of its engines on Boeing 787s and 787-8s — software to better detect the icing in the engines and to open a duct that ejects ice from the center of the engine, changes to the shape of the housing for a temperature sensor in the air flow path where ice would build up, and new compressor blades and inlet vanes. One hurdle to more progress has always been a lack of knowledge about the precise characteristics of the partially melted ice crystals that are most prone to causing dangerous accretion. Without that data, computer models can’t precisely predict the weather conditions that will cause the kind of crystals that turn into dangerous drops or tell engineers how best to design new engines that will defeat the icing.
This is the story of how the Penn State team came up with a measurement technique that could clear the way for more anti-icing innovations to come.
Building a model
The Penn State researchers were motivated by a desire to help NASA run its high-altitude icing wind tunnel experiments with maximum accuracy when testing engine components. In a series of modifications from 2012 to 2015, NASA upgraded test chambers at the Propulsion Systems Laboratory at the Glenn Research Center in Ohio to replicate the low temperature and pressure conditions of high-altitude icing. The trouble is, turning on the nozzles to spray liquid droplets changes the temperature and humidity as the droplets partially freeze before hitting their test surfaces, which could be ducts or the non-moving blades in the compressor section. That makes it especially difficult to set the exact conditions to create partially frozen ice crystals with a specific ice-liquid ratio that hit the surface at a speed typically between 85 and 135 meters per second, says Tadas Bartkus, a senior research associate with the icing branch of the Ohio Aerospace Institute, a NASA contractor.
It’s a tricky environment for the researchers to set up correctly. “In general, you see a one- to two-degree change and you think it’s not that big a deal. But not when it comes to icing.”
Bartkus needed to verify the accuracy of a computer model he created to predict the complex interactions in the icing wind tunnels. If he could make the model accurate enough, testers could calculate the wind tunnel conditions they would need at the beginning of the test to arrive at the conditions they wanted at the end. So NASA asked the Penn State team to assist Bartkus. He needed test data on partially melted ice crystals to help verify assumptions in the computer model about how long it takes them to melt at specific temperatures and how that time changes for different crystal sizes.
Luckily, in 2015 while working on another project, the Penn State researchers had found a method of creating partially melting crystals and measuring their temperatures and ice/water ratios without touching them. The technique had taken two years of trial and error. At first, they tried shooting ice crystals from a pneumatic gun and passing them through a heating chamber before the partially melted crystals hit a test surface designed to mimic an engine component. But the crystals traveled at 100 meters per second and didn’t have time to melt properly. The same problem resulted when they shot crystals through the flame of a blowtorch; it was like quickly passing your hand through a flame, Palacios says. Next, they tried something more complex. They froze a drop of water by letting it fall through a liquid nitrogen-cooled tube, then partially melting it by passing it through a heating chamber before blowing it with air toward the test surface. Nothing worked until they tried an entirely new approach. They started with a drop of liquid water and suspended it in midair with acoustic waves created by an ultrasonic levitator. They blew cold air on the droplet to freeze it, and then stopped the cold air and allowed warm air to partially melt the drop. This was the approach they would take with the validation testing for the Bartkus computer model in 2016.
Another problem was how to measure the changing amounts of liquid and frozen water in the drop — a key measure for the NASA computer model to show how fast and how much the drop melted under specific conditions. The researchers accomplished that with another method they had developed previously. They infused each drop with Rhodamine B, an ink that glows orange in liquid water when hit by green laser light. Ice does not react to the laser. They compared the laser-lit water layer to the dark ice core in magnified photos.
Bartkus tweaked the computer model, which he has been writing and refining for about two years, based on the Penn State test results for the levitating partially melted crystals. The Penn State results improved the accuracy of the model’s assumptions about how the crystals melt under specific conditions they encounter in the PSL wind tunnel. Now, Bartkus is working on improvements to the model so it can more accurately predict test conditions when the spray nozzle droplets are not uniform throughout a given cross-section of the icing wind tunnels.
The Penn State team members continue to study the fracture and splashing dynamics of partially melted crystals. Their tests could help explain why some of the crystals don’t shatter, which might be because their watery exterior has a cushioning effect. The tests might also help define the crystal-liquid ratios that create the most freezing after impact. This is the phenomenon they had attempted to test starting in 2013 before they had settled on the acoustic levitator idea. Now, with a stationary partially melted ice crystal, they smash the ice-liquid mix with a moving surface — the pneumatic bullet — to simulate a fast-moving airplane engine. By lighting up the dyed, partially melted ice crystal with the green laser, they can precisely measure its ice and liquid volume before impact.
The pneumatic bullet tests help answer two questions, Palacios says: Are the predictions of fracture dynamics models correct? And how much ice freezes to the impact surface as the ice-liquid mix varies?
“If you can validate these values in the models, then you can have some certainty that your predictions for fracture dynamics are somewhat correct and then you can use these models to model more complicated circumstances,” Palacios says. As for how much ice freezes to the surface, the answer to that question can form the basis for new computer models that will predict icing for specific types of partially melted crystals.
If all goes as planned, jet-engine manufacturers could have a computer model to virtual-test their designs for icing risk, instead of building engines and then testing them in wind tunnels. Also, engines designed from the ground up with the specific icing scenarios in mind would be more fuel efficient, powerful and reliable than engines that were retooled after the fact to avoid icing flameout.
“It would be very expensive and challenging to do these fundamental tests on a full-scale engine, rather than just telling you if this engine is going to fail or this engine is going to pass or you have this performance reservation on the engine” based on computer simulations, Palacios says.
Editor’s note: In the Penn State University photo at the top of this webpage, ice accretions on an airfoil were recreated in the Propulsion Systems Laboratory at Glenn Research Center as part of research to understand the physics of icing that can occur inside jet engines.
Dangerous crystals
Deep convective thunderstorms contain ice crystals that flow out from the tops of billowing clouds. These crystals are all but invisible, and pilots are instructed to give such storms a wide berth. Still, an aircraft’s engines sometimes ingest crystals.

About Keith Button
Keith has written for C4ISR Journal and Hedge Fund Alert, where he broke news of the 2007 Bear Stearns hedge fund blowup that kicked off the global credit crisis. He is based in New York.
Related Posts
Stay Up to Date
Submit your email address to receive the latest industry and Aerospace America news.
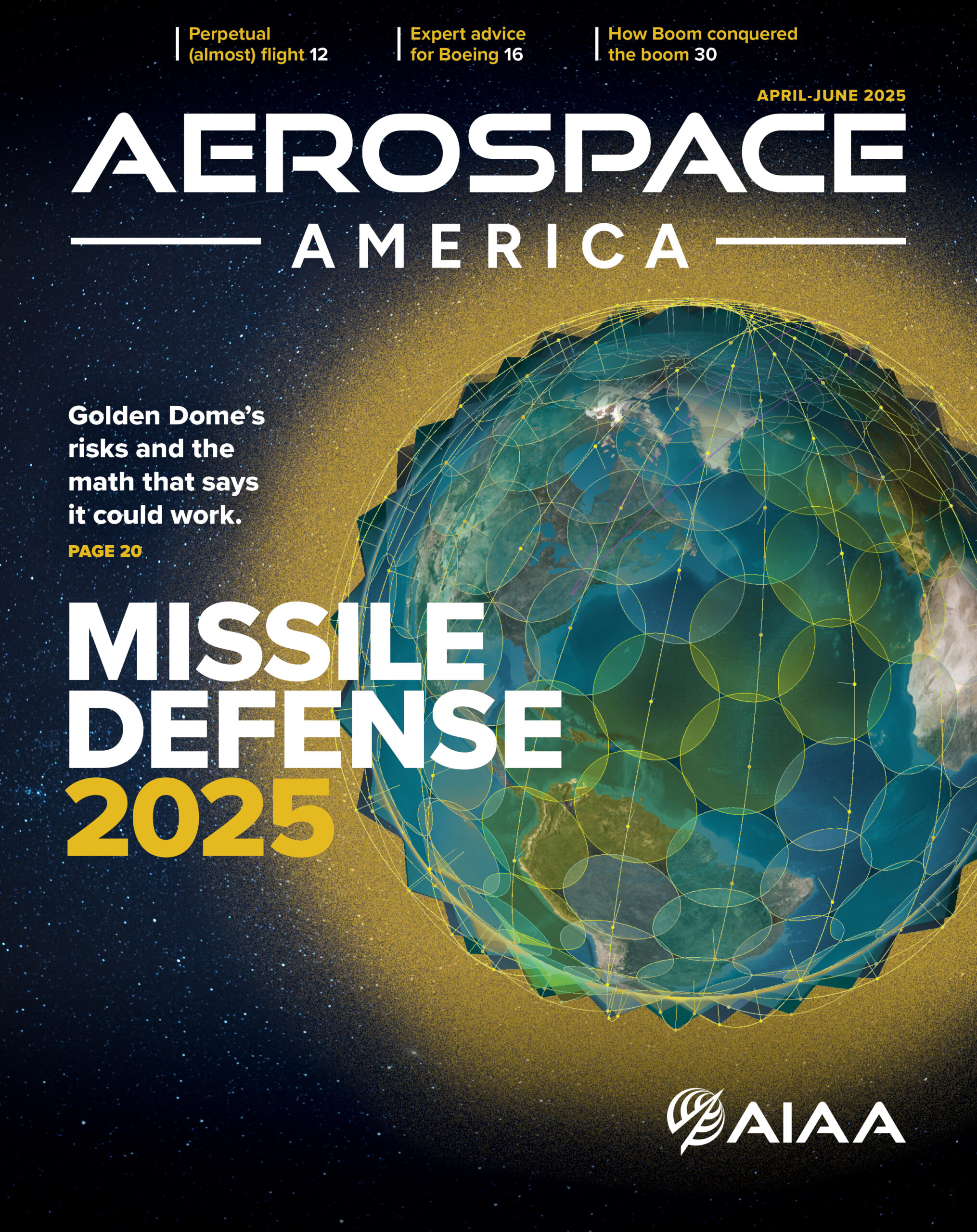
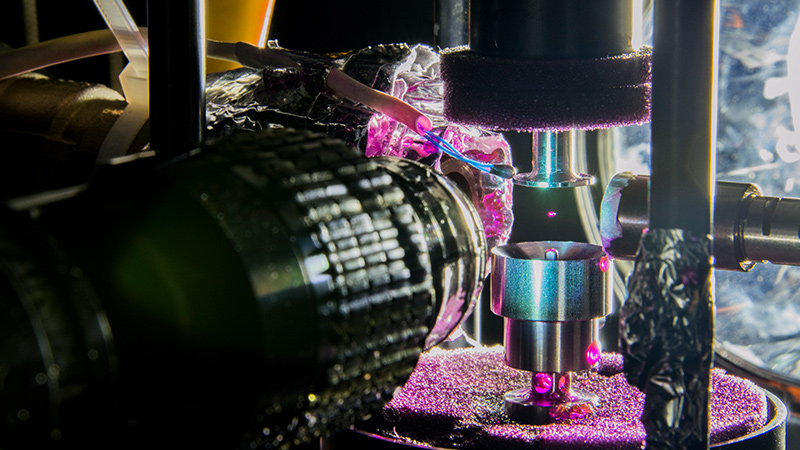