Stay Up to Date
Submit your email address to receive the latest industry and Aerospace America news.
During the years NASA has been planning its Mars Sample Return mission, coders were busy charting an artificial intelligence revolution, while robotics engineers continued making ever more sophisticated machines. Is it time to consider analyzing the samples on Mars instead of bringing the rocks and dirt home to search for organisms or evidence of ancient life? Jon Kelvey asked the hard questions and learned the harsh realities.
Theory holds that about 3.7 billion years ago, a meteor crashed into the equatorial region of Mars, cracking its surface and leaving a hole nearly as wide as Lake Michigan into which water seeped from below and melted snow flowed in from the sides, as NASA describes the scenario. The lake is now a dry crater, but the timing of this feature’s watery phase has long intrigued scientists. Life arose on Earth about 3 billion years ago, an era when Gale Crater was still a lake, scientists believe. Perhaps life emerged there, too, and the sedimentary rocks in the crater hold records of it. So in 2014, NASA’s Curiosity rover drilled into a piece of hardened sediment — mudstone — to look for chemical markers of ancient life.
Inside its chassis, Curiosity heated the drilled material to 860 degrees Celsius in an oven to release fumes from it that were fed into the onboard mass spectrometer. The constituent particles were given an electric charge and sent through a magnetic field that deflected their trajectories by an amount corresponding to their masses. From those masses, the likely presence of particular molecules could be deduced.
The results hinted that the mudstone might contain kerogen, a complex organic compound that can be found in lifeless meteorites, but also, tantalizingly, “at least on Earth, is a leftover from the decay of plants,” explains physicist Scott Hubbard. As head of NASA’s Mars science program in the early 2000s, he restructured the effort partly toward bringing samples to Earth for analysis. On Earth, layers of kerogen were formed from deposits of algae, plant pollen and plankton in mud and underwater sediment that were squeezed by geological forces. Gale Crater was exactly the sort of place one might expect the Martian version of this process to occur, but there was a hitch: One technology was best suited for conclusively determining whether the material contained kerogen — and if it did, whether its origins were biological — but this technology was back on Earth in any of various massive particle accelerator facilities around the world.
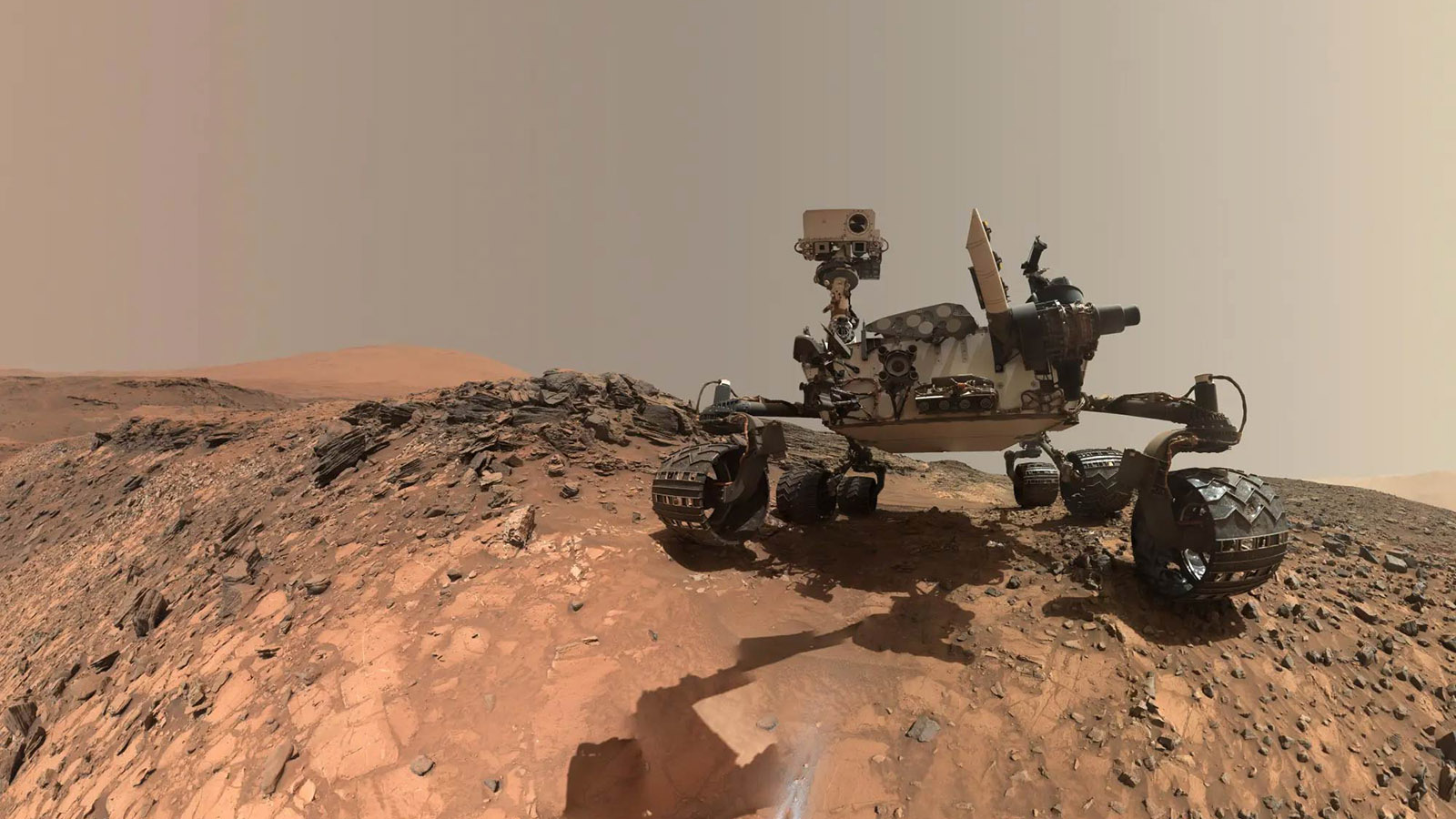
My interviews with a dozen Mars scientists, lunar scientists and engineers show that the large size of the most capable technologies for addressing the question of life on Mars is not driven by a lack of intelligent computer code or microprocessors (as valuable as those could prove to be) but by the physics of fully analyzing samples. The size issue is true for an electron microscope that could in theory capture fossilized microbes, but whose dimensions are typically measured in meters. It is most apparent when employing synchrotron radiation, a form of X-ray light that must be generated in a particle accelerator. If directed at a mysterious material, such as the core sample Curiosity drilled up, the X-rays would reveal unprecedented detail.
This size issue, more than any other, is why NASA so far has not wavered from its goal of bringing samples home, even as scientists continue to deliberate over specific science objectives and the agency searches for alternative mission architectures that could tame Mars Sample Return’s escalating costs. The mission was projected in 2020 to cost $4 billion, but the estimate has since soared to $8-$10 billion, according to the September report from the Mars Sample Return Independent Review Board 2, the latest group to review the mission.
Under the current plan, samples would be brought home from Jezero Crater, a feature similar to Gale Crater in that it is also thought to have once been a massive lake but is now dry and might contain kerogen or other signs of Martian life. Curiosity’s successor, the Perseverance rover, arrived there in 2021 and has been drilling samples and inserting the material in cigar-sized titanium tubes. Some of the tubes have been left on the surface for possible later collection, while others are riding on the rover. It’s the next phase, in which those tubes would be collected and brought to Earth, where adjustments to the architecture are possible. The required Mars surface hardware has yet to be built, and the review board warned that existing plans cannot “be accomplished with the likely available funding.” NASA responded by assembling an interagency team that’s supposed to propose alternative mission designs by March. Congress has also taken note, with the Senate Appropriations Committee threatening in draft budget language to descope or cancel the mission if total anticipated costs are not brought down to $5.3 billion.
The existing mission design includes dozens of steps requiring coordination among as many as seven spacecraft of various kinds: Perseverance is to navigate to a U.S.-built lander, whose robotic arm would grab the tubes from the rover and place them in a protective sample container. NASA designed in a contingency plan, however, in case Perseverance is no longer operating when the lander arrives at Mars in 2030. In that event, two small helicopters would be dispatched to retrieve the tubes. Once the tubes have been collected, a small U.S.-built rocket would blast off from the lander to boost the sample container into space, where it would be released and captured by a European-built Earth Return Orbiter. Once near Earth in 2033, the orbiter would release the container to enter the atmosphere for a landing under parachutes.
No one I spoke to suggested that a switch to analyzing the samples on the surface of Mars could match the quality of science that could be done by bringing samples to Earth and blasting them with X-rays or scanning them with an electron microscope. But one scientist said interesting science could be done in situ, even if not a direct replacement, and that costs and benefits of returning samples must be carefully weighed.
“For the price of one Mars Sample Return mission, you can do at least three solid in situ exploration missions,” says Jorge Vago, project scientist for the European Space Agency’s planned ExoMars mission, a separate endeavor in which a small rover would land on Mars in the late 2020s. “The cost of a Mars Sample Return class of mission must be justified by what you can learn with the material that you will bring back. So you need to be sure that what you return to Earth is worth it.”
Ultimately, he says, “With a robotic mission we have — at present — no hope of being able to image putative microorganisms. We could see colonies, perhaps, but not the individuals.” For instance, “we cannot make thin rock slices and image them with an electron microscope as we would on Earth.”
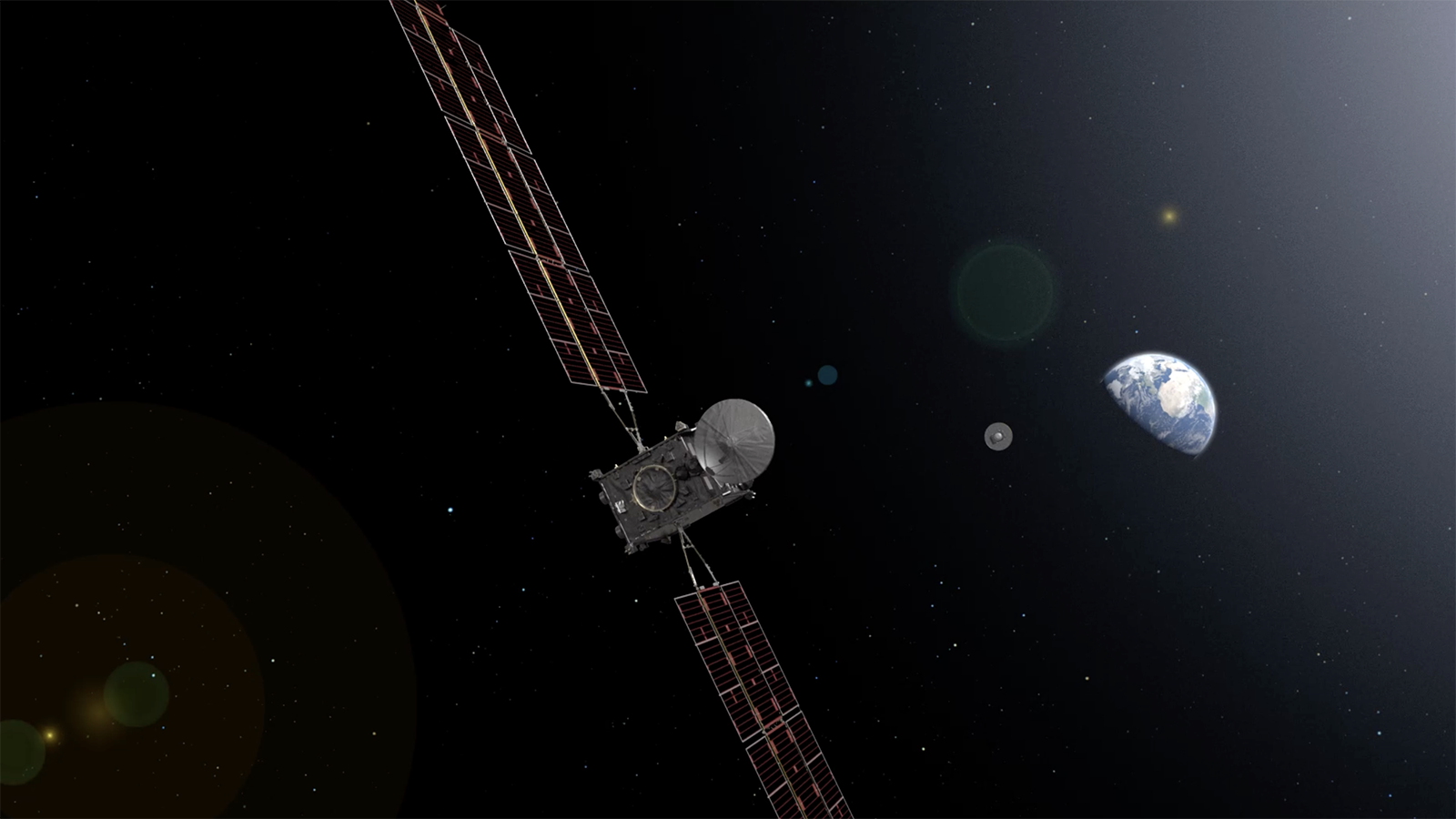
Likewise, Susanne Schwenzer, associate director of astrobiology at The Open University in Milton Keynes in the U.K. and a member of the Mars Sample Return Campaign Science Group, believes that “if you take Curiosity one, five, 10 steps further, I think there would be a lot that we can do” in situ. But she, too, is not suggesting that such analysis could match the science that could be done if MSR succeeds in returning the samples.
For one thing, only so many instruments can fit on a rover. And no matter how advanced those instruments might be, Schwenzer says, you wind up with new, ambiguous discoveries that cannot be cleared up with the instruments on hand. “If you send a spacecraft, you’ve got a set of tools,” she says. “And you can’t exchange the toolbox.”
Take NASA’s first landings on Mars, the Viking 1 and Viking 2 landers in 1976. Scientists at that time hoped to find out whether there were microorganisms on Mars, but they had no direct way to search for them. So they devised a “labeled release” experiment in which nutrients labeled with radioactive carbon-14 were added to Martian soil. If organisms were present, they would consume the nutrients and release carbon dioxide waste gas containing carbon-14. The results looked promising for the presence of organisms, but later were determined to be debatable.
“At the time they devised the instrument, we did not know that the Martian soil often contains perchlorate, which could mimic the ‘biologic’ reaction,” Schwenzer told me by email. “The power of the return samples is you go, ‘I found this unexpected thing! Now my colleague in Japan has a specialized instrument; why don’t I get the sample to him or her?’”
The discovery that soil often contains perchlorate had to wait for NASA’s Phoenix lander to arrive in 2008 — 32 years after the Viking landings. As important as that discovery was, solving other mysteries such as the one about kerogen cannot today be done by instruments of rover scale. “Synchrotron radiation is a great example of something the size of a football field that you’re not going to be able to put on a spacecraft,” says Michael Meyer, the lead scientist for NASA’s Mars Exploration Program. In fact, he jokes, “it’d be scary if you could put that on a spacecraft.”
And the size and weight are just one part of the problem: “Viking taught us that there’s not a single measurement that’s going to answer your question of whether or not there’s life elsewhere. It has to be in context.”
Over the years, NASA’s Mars program has slowly built up a contextual understanding of the geologic history of Mars. The Sojourner rover in the late 1990s and the twin Spirit and Opportunity rovers in the early 2000s helped establish that liquid water once flowed on the surface. Curiosity established that conditions on early Mars were ripe for evolution of life. “It had oxidized and reduced species. It had organic compounds,” Meyer says.
Meyer views MSR as the next logical step in NASA’s Mars science strategy. The rocks and dirt could be stored over the decades, he points out, just as samples from the Apollo program continue to yield new discoveries 50 years after the program ended.
“A decade from now, [scientists] can ask a question nobody thought of,” he says. “They can use instrumentation that hasn’t been invented yet.”
While the architecture review progresses toward the March deadline, MSR scientists are at least partially in limbo in their planning. As central as the search for life (past or present) is to MSR, it is not the only priority whose details need to be settled. In 2018, the International MSR Objectives and Samples Team listed the search for life among other objectives, including interpreting the geological processes that created Mars, with a focus on water; determining what resources exist to support future human exploration of Mars; and identifying hazards to those explorers.
Just how to meet those objectives, which questions to ask with which instruments, testing which samples in what order, and storing them on the ground in what and where, is subject to ongoing debate among the scientists within the MSR Campaign Science Group.
“We are having very, very long discussions about these things,” says Schwenzer, a member of the group. “For example, you take the headspace gas out of these sample tubes, what do you replace it with? Nitrogen? Argon? Helium? Something else entirely? Depending on whom you ask, the answer is different.”
Optimizing something as simple as which neutral gas to use for storage is complicated because there are contrasting and even conflicting requirements for the different MSR science objectives. The samples must be stored under very dry conditions to avoid any terrestrial moisture seeping in and contaminating the material, according to Schwenzer. But that same dry atmosphere poses a challenge for preserving any hydrated matter, such as gypsum, a residue of saltwater evaporation, that might be on Mars. This drying could interfere with organic chemistry experiments “where we have time-critical measurements to make,” she says.
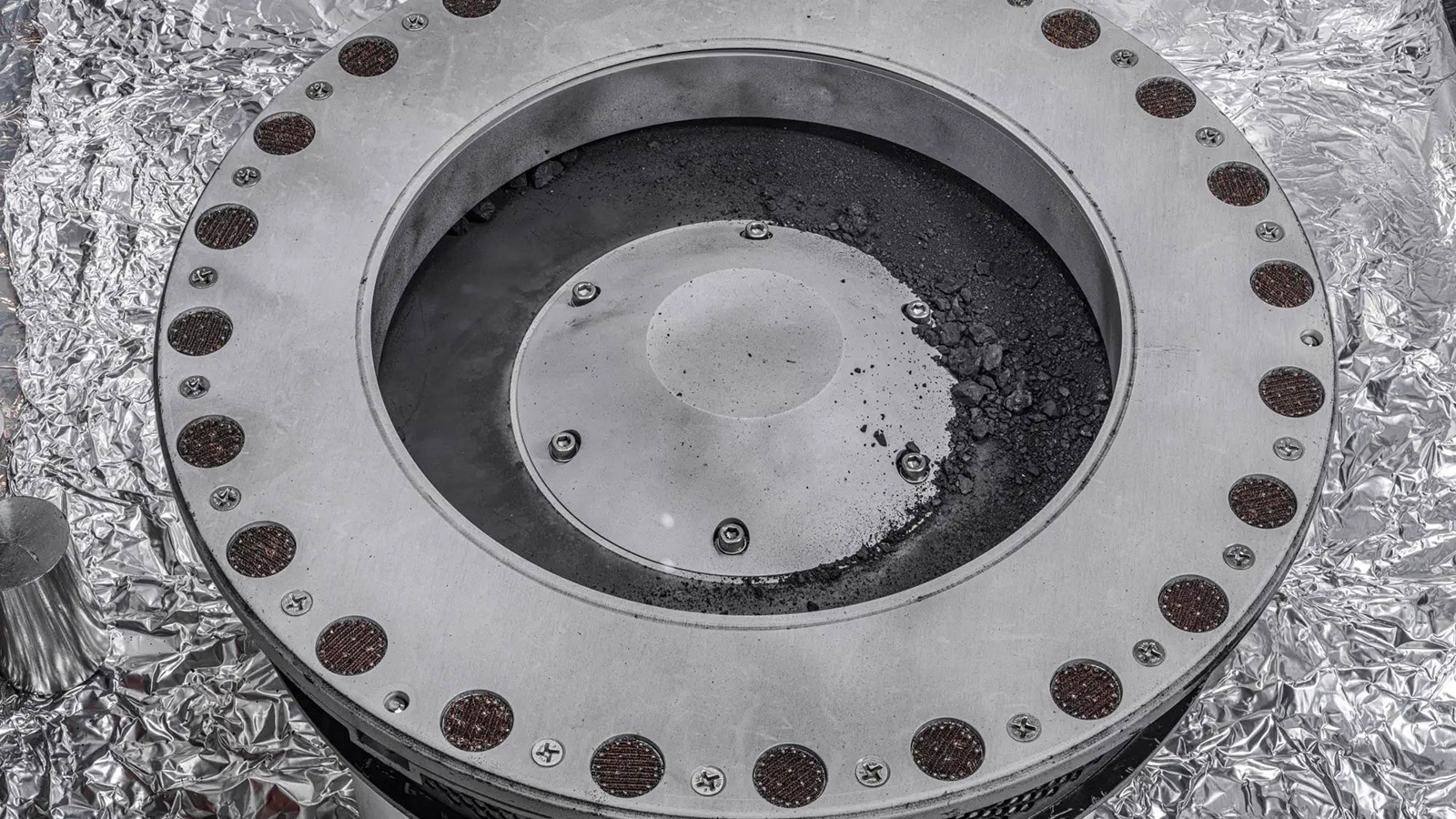
There are similar tensions for determining at what temperature to store the Mars samples in order to facilitate both geological and astrobiological experiments. “If you really have something alive, minus 80 [Celsius] is the way to go to preserve it. That’s what the biologists are telling me,” Schwenzer says. But store something with water in it at minus 80, and it will develop cracks; “take it out and put it back into that cold, and you’ll get freeze-thaw process damage,” which could hinder mineralogical studies of the Mars material.
Also, the possibility of the samples containing still extant Martian life, however remote, introduces additional challenges not faced, for example, by the OSIRIS-REx team that last year brought back samples from the asteroid Bennu. The Mars samples can’t be transported to a conventional clean room but must be held in a biocontainment level 4 facility, the same level of biosecurity used for the U.S. Centers for Disease Control and Prevention lab studying the smallpox and Ebola viruses. No such NASA facility exists at the moment, and that could be a problem.
“A lot of things that need to happen need to happen now. We need to build a receiving facility now,” Schwenzer says. “These things take 10 years to build.”
For now, the various teams of scientists are “on standby, almost, waiting for a better signal for what’s the next thing they need to pay attention to, what’s the next thing they need to work on,” says David Beaty. He’s a petrologist at the NASA-funded Jet Propulsion Laboratory in California and acting lead scientist for the sample receiving project, part of an office NASA opened early last year to manage the receiving and curation of the Mars samples.
There is some work still going on. For instance, the Measurement Definition Team, another set of international Mars scientists, is working on a report detailing which instruments should be included in the sample receiving facility, says Beaty. This report, also due in March, will provide the list of required instruments and the science and technical staff needed to operate them.
“We want the smallest number of instruments that we can and still do the job correctly,” Beaty explains. “It’s a pretty big driver on the sizing and therefore costs of the sample receiving facility. And we need to know that relatively soon.”
As for when scientists might get their figurative hands on the samples, an air of realism has set in.
“I’m far enough along in my career that I don’t have hope anymore that the samples will arrive in my professional lifetime. I worry more that they arrive in my physical lifetime,” says Beaty, who started his career working with lunar samples from the Apollo program.
Despite the challenges ahead, Hubbard believes that MSR will go forward, one way or another.
“It is my belief that, like [the James Webb Space Telescope], NASA will find a way — with Congress — to fund MSR,” he says. “The science return, possible fingerprints of life, is of the highest priority.”

About jon kelvey
Jon previously covered space for The Independent in the U.K. His work has appeared in Air and Space Smithsonian, Slate and the Washington Post. He is based in Maryland.
Related Posts
Stay Up to Date
Submit your email address to receive the latest industry and Aerospace America news.
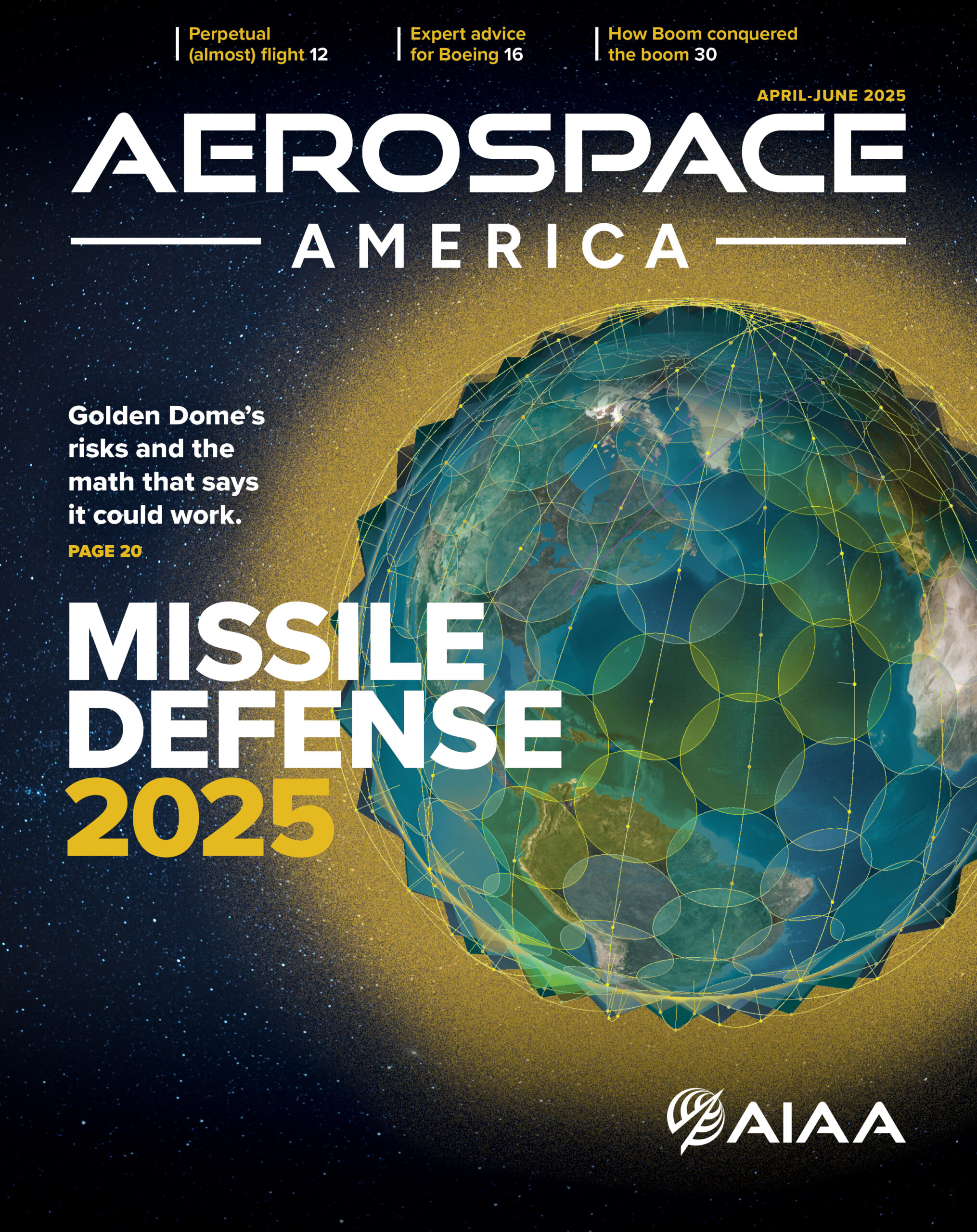