Stay Up to Date
Submit your email address to receive the latest industry and Aerospace America news.
NASA is testing technologies to bring nuclear fission power to human spaceflight. Former astronaut Tom Jones explains why the move is long overdue.
In space, electricity is life. Remember the Apollo 13 crew’s brush with death when an oxygen tank explosion took out their power-generating fuel cells? Or perhaps you’ve heard how in 1997 astronaut Mike Foale, working on the Mir space station with his two cosmonaut hosts, witnessed the lights go out in his orbital home after a cargo ship collision tumbled the station and pointed its solar arrays away from the sun. The powerless station drifted in the cold of space for over a day until the crew, armed with flashlights, fired up their Soyuz thrusters to coax the arrays back into sunshine. Without electrical power, survival in space is impossible.
Photovoltaic solar arrays worked well on Mir, and they work well on the International Space Station, but they can’t convert enough sunlight to electricity to meet the sizeable energy needs of human explorers beyond the moon, or on a planetary surface. Neither can radioisotope thermoelectric generators, or RTGs, like those that powered most of Apollo’s deployed lunar surface science packages and that have powered robotic probes heading for regions where sunlight is too weak for solar arrays.
Deep space explorers are going to need a fission reactor, probably a collection of them. Unfortunately, for decades NASA limited its in-space production of nuclear-generated electricity to RTGs, judging fission reactors as too expensive and politically sensitive to develop. Besides, with astronauts limited to low Earth orbit, solar energy sufficed to power the ISS.
Now, NASA is factoring fission reactors into its human exploration plans, and under a project called Kilopower it is laying the groundwork for a future flight demonstration of the technology.
This is long overdue. Fission reactors can be built large enough to not only generate electricity, which is Kilopower’s role, but also to drive an efficient rocket engine. For propulsion, the nuclear reaction can supply heat to generate electricity for ion engines or, at higher power levels, blast tons of high-speed propellant out a rocket nozzle. A high-thrust nuclear thermal rocket can haul bigger payloads for the same propellant load or lower the trip times for missions to the moon or Mars. The U.S. flew an electricity-generating, 600-watt fission reactor, SNAP-10A, in 1965. The Soviet Union launched at least 30 small reactors into low Earth orbit to provide electricity for military radar reconnaissance satellites. But these low Earth orbit satellites suffered from failures that dumped a pair of their reactor cores in the ocean and in 1978 scattered another’s radioactive debris across Canada. NASA will have to design future reactors to survive launch accidents intact and begin operations only once they are well away from Earth.
By the early 1970s, NASA had ground-tested a series of nuclear thermal rocket engines at the Nevada test site; these systems generated up to 4,500 megawatts of thermal power, produced 1.1 million newtons (250,000 pounds) of thrust, about half the thrust of a space shuttle main engine, and accumulated 90 minutes of run time. Unfortunately, this promising NERVA, for Nuclear Engine for Rocket Vehicle Application, space propulsion system was discarded during the agency’s precipitous post-Apollo downsizing.
Yet to explore deep space, NASA really has no choice but to get serious again about fission. Solar power has drawbacks: The ISS requires an acre of solar arrays and a massive battery suite to support six astronauts. On the red planet, dust-obscured solar panels spelled doom for Spirit and Opportunity, NASA’s two Mars Exploration Rovers. At Mars, solar power is further compromised, because sunlight there is only half as intense as at low Earth orbit or on the moon. Supplying a Mars outpost by solar power alone is a marginal proposition at best.
Nuclear fission offers distinct advantages in supplying the power-intensive needs of human explorers. Nuclear is always on, day or night, eliminating batteries. Reactors are also compact and immune to obscuring dust. And their ample power output gives deep space crews a higher margin of safety.
On the moon or Mars, a compact fission reactor could be landed, deployed and connected robotically before a human crew arrived at an outpost. Fission could supply ample “juice” for drilling, heating, cryo-chilling, materials processing, additive manufacturing, communications, rover recharging and sample collection. Steady, abundant electricity will be essential to produce an expedition’s Earth-return propellant from subsurface ice, or at Mars, atmospheric carbon dioxide.
NASA’s Kilopower
Kilopower is aimed at developing concepts and technologies that could be used in an affordable fission power system supporting long-duration stays on planetary surfaces. Managed by NASA’s Game Changing Development program within the Space Technology Mission Directorate, Kilopower kicked off in 2015, and in less than three years, and for under $20 million, NASA and its government agency partners designed, assembled and operated a uranium-235 fission reactor to produce 10 kilowatts of power under realistic space environmental conditions.
Kilopower’s output is small compared to the 84-120 kW produced by the ISS’s solar arrays, which could power about 40 homes. Kilopower’s 10 kW cannot even match the steady 21 kW produced by my shuttle orbiter’s three fuel cells. But it could be networked with several identical reactors to create a surface electrical grid, available 24/7. Nuclear could supply a lunar outpost, for example, with all the electricity needed for life support, communications, active thermal control, rover recharging and propellant production.
At Kilopower’s heart is a cylindrical, solid-cast core of uranium-235 about the size of a roll of paper towels. A chain reaction begins only when a cylindrical shell of neutron-reflecting material slides over the core, generating heat then carried by passive sodium heat pipes to Stirling energy converters. These efficient piston engines have run continuously for a decade or more in lab tests, powered by heat from non-fission sources. The electricity they generate is delivered to users while excess heat is dissipated by passive radiators. Kilopower’s core is self-regulating: As heat causes the metallic neutron reflectors to expand, they trap fewer fission neutrons, thus slowing the chain reaction.
Fission reactors are of course radioactive, and in space applications the designers must consider hazards not present in solar or fuel cell designs. The fissile material must be protected and contained in case of a launch accident, but Kilopower’s U-235 core carries fewer than 5 curies of total radioactivity while inert on the launch pad. That’s less than a typical plutonium RTG. By comparison, a hospital’s radiotherapy machine might contain as much as 1,000 curies of a potent radioisotope.
To limit ground hazards, reactor operations would not commence until the unit was outbound from Earth or delivered to a planetary surface. Once there, regolith berms or burial would protect crews and equipment from radiation. Finally, Kilopower is designed to preclude any chance of a meltdown: Loss of cooling would cause a thermal expansion and an automatic reduction in reactor power.
KRUSTY delivers
To test these design features, NASA and its partners — the Department of Energy, the National Nuclear Security Administration, the Los Alamos National Lab, the Y-12 National Security Complex and the Nevada National Security Site — ran a 2018 test called KRUSTY, for Kilopower Reactor Using Stirling Technology. KRUSTY aimed to show the Kilopower system could generate fission electricity and remain stable and safe under space-relevant environmental conditions.
At the Nevada test site, the Kilopower reactor generated a controlled chain reaction, generating from 1 to 10 kW of thermal power while exposed to realistic operating temperatures in a vacuum. After normal operation was achieved, engineers introduced off-nominal situations, such as a failed Stirling converter or failed heat pipes, that might overheat the active reactor core. Kilopower self-regulated its output and maintained safe operations.
Finally, the reactor was put through a 28-hour, full-power demonstration of start-up, ramp-up to full power at 800 degrees Celsius, stability under introduced transients, and a commanded shutdown. NASA Glenn’s Marc Gibson, Kilopower lead engineer, said last July in a statement that “the reactor performs very well. … We were able to make the fuel and test the reactor here in the United States using our existing facilities. … I think that gave NASA management a lot of confidence that this isn’t just a paper study — this is real.”
Although the KRUSTY configuration was not a flight system — the reactor lacked a radiator, a full suite of Stirling engines and a startup control rod, and was not subjected to launch loads, flight vibrations or free fall — its performance was an encouraging first step toward practical space fission power.
Next: fission propulsion
The ultimate application of fission power is high-thrust space propulsion, escaping the limitations of chemical rockets. NASA let a contract in 2017 with the nuclear-engineering firm BWXT to examine design and licensing requirements for a full-scale, full-thrust ground test of a nuclear thermal propulsion engine — along the lines of the NERVA tests 50 years ago. The $18.8 million contract includes the conceptual design of a propulsion reactor that, because of its much higher rocket exhaust velocity, would be about twice as efficient as chemical rockets such as the LOX/hydrogen space shuttle main engine. Such a 500-megawatt (a measure of reactor power) nuclear thermal rocket might have a low-enriched uranium core to alleviate proliferation security concerns and ceramic/metallic fuel elements to deliver a specific impulse, a measure of fuel efficiency, of 900-910 seconds (compared to the shuttle main engine’s 450 seconds). Such an engine could halve a Mars expedition’s propellant requirements, or greatly increase its payload. Similarly, efficient nuclear propulsion can shorten one-way Mars cruise duration from six months to four, sparing astronauts excessive exposure to space radiation and free fall.
Such an engine is a couple of decades in the future, and making it a reality will not be easy. It will demand courageous political leadership at NASA as much as crackerjack nuclear engineering. But the success of Kilopower is a heartening sign of cold-eyed, purposeful management. As Glenn’s Marc Gibson put it: “We’ve got a team that can go and design, test and build nuclear for space. We can go and use this technology on missions. That’s the piece that hasn’t been there in the last 50 years — no one ever tested the things that they put on paper.”

About Tom Jones
Tom flew on four space shuttle missions, including his last flight, STS-98, in which he led three spacewalks to install the American Destiny laboratory on the International Space Station. Tom is a senior research scientist at the Florida Institute for Human and Machine Cognition.
Related Posts
Stay Up to Date
Submit your email address to receive the latest industry and Aerospace America news.
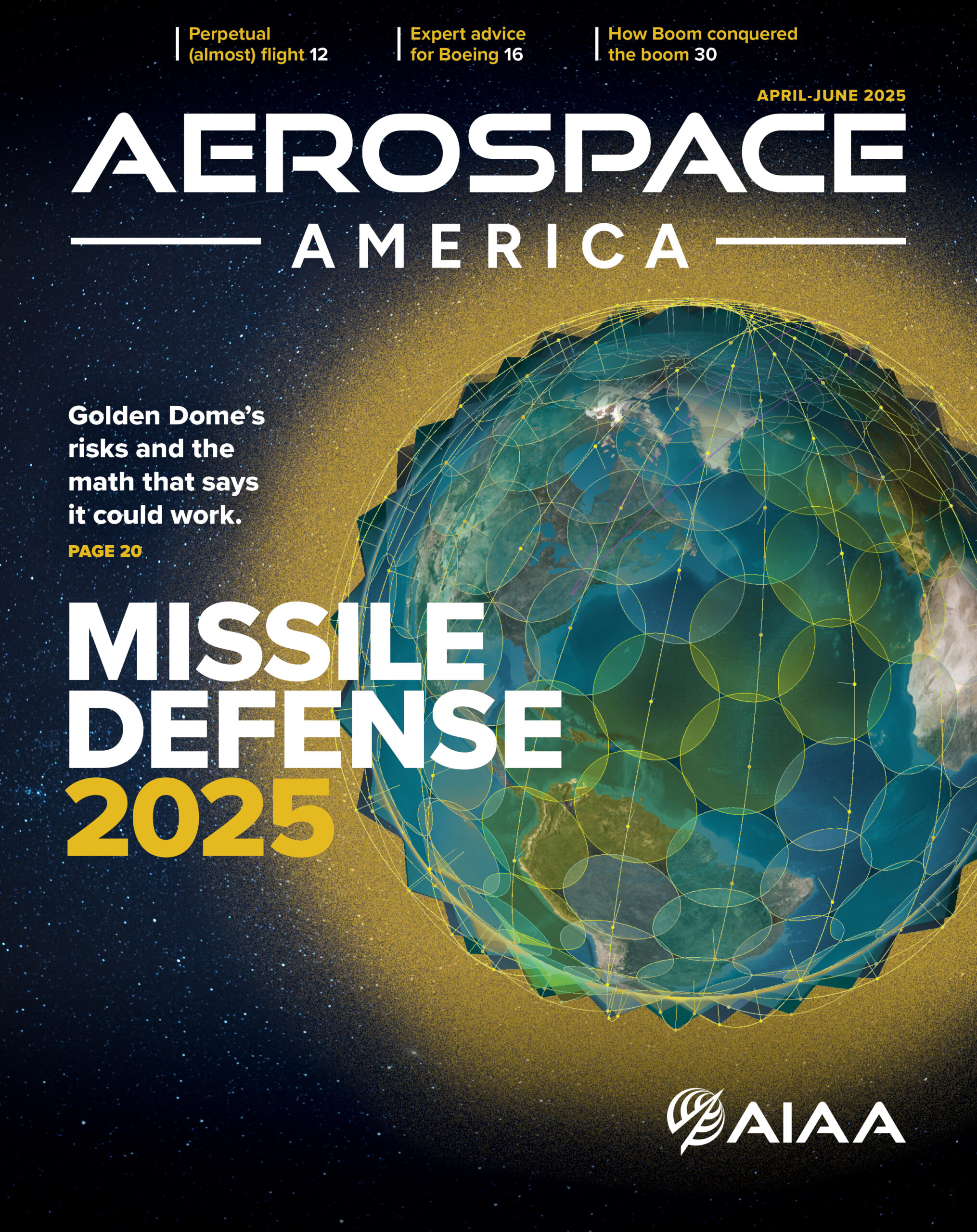
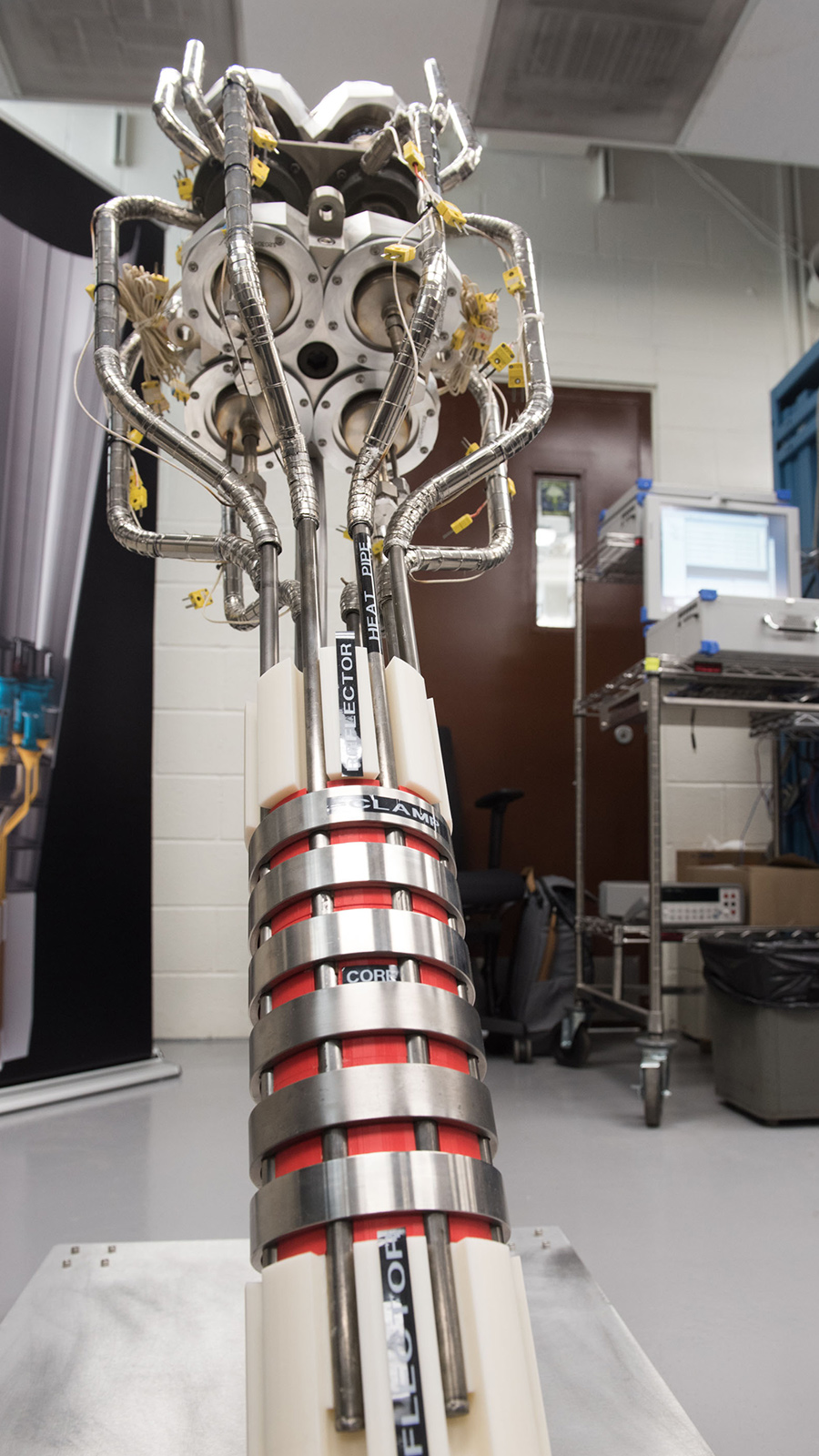
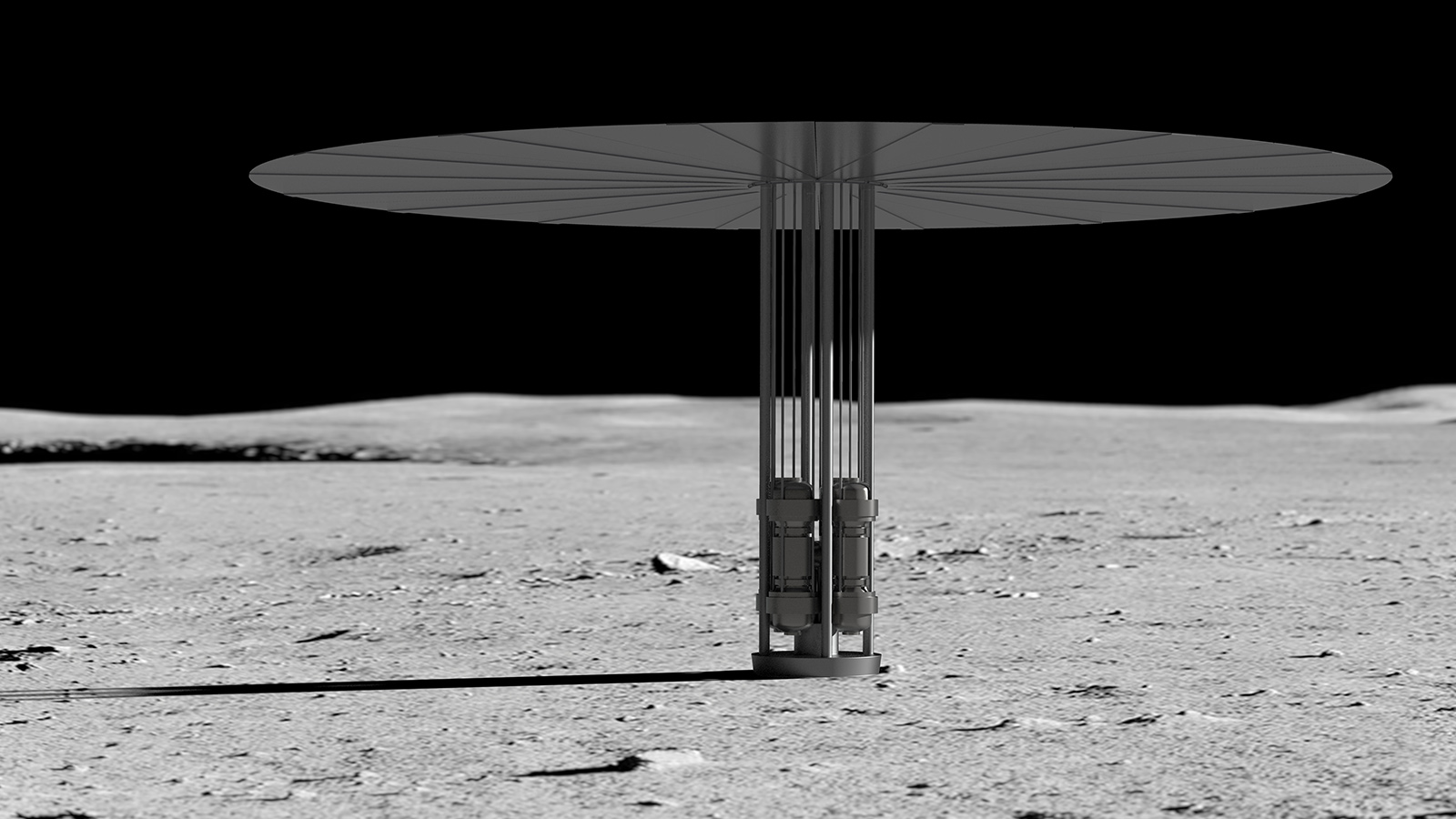
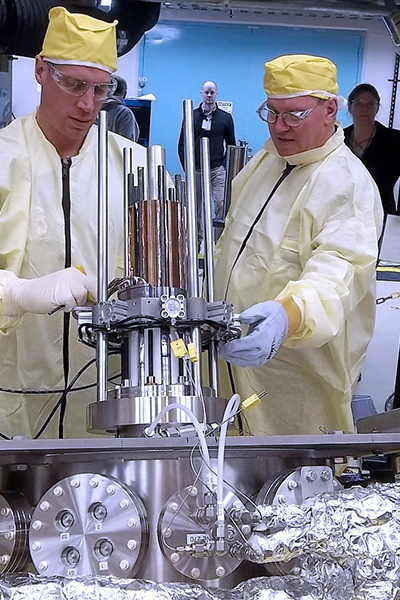