Stay Up to Date
Submit your email address to receive the latest industry and Aerospace America news.
NASA and university researchers could be on the cusp of a new structural health monitoring approach for aircraft and spacecraft. The technique is centered on a two-part composite material that emits light when flexed. Keith Button spoke to the developers of the mechano-luminescent-optoelectronic, or MLO, composite.
Part of Alexander Chin’s job at NASA is to evaluate new technologies. He admits to being a bit skeptical two years ago when a colleague told him about a newly invented material that glowed and produced electric current when damaged.
The flexible material consisted of two polymers: a conductive polymer with a photoactive layer and an elastomer embedded with crystals. The crystal-embedded component glowed green or orange or blue, depending on the formulation, and the other polymer component reacted to this glow by generating an electric current. The marriage of the two materials was invented about five years ago by Donghyeon “Don” Ryu, an engineer in the growing field of smart materials who at the time had just joined New Mexico Tech, where he is an assistant professor. He called his rubbery creation the MLO composite, short for mechano-luminescent-optoelectronic composite. He has a patent pending on the invention.
Ryu showed Chin photos and some of the results of preliminary tests on the polymer composite. The data showed that the electric current was measurable and that the material was sensitive to strain as well as damage.
Chin, who works at the Armstrong Flight Research Center in California, encouraged Ryu to apply for NASA grants.
Now, after a year of NASA-sponsored lab testing on versions of Ryu’s MLO composites, Chin considers the technology promising. With more testing, it could prove to be worthy of pushing up NASA’s scale of technology readiness levels, he says.
Researchers at the center’s flight loads laboratory will soon install a 60-by-90-centimeter section of an MLO-equipped wing built by Ryu onto a heavy bench for a series of tests to be supervised by Chin. Starting in June or July, Ryu’s team will shake the wing section to find out how sensitive the material is to the flexing and bending that results from a wing vibration. The data produced by the MLO composite will be compared to that from traditional strain and damage sensors, such as accelerometers and strain gauges.
The results could point to a lighter, more sensitive technique for warning when a wing or other structure of a conventional aircraft or drone or spacecraft is enduring excessive wear or at risk of breaking apart. Embedded as a layer within the entire skin of a wing or fuselage, connected by electrodes to a computer reading its voltage output, for example, the MLO composite could serve as a sensor operating under its own electric power. With the added sensitivity, operators might be able to fly their aircraft harder. And with a reliable, precise sensor, designers would have the freedom to create new designs for more fuel-efficient aircraft. They could build lighter aircraft, with more ambitious designs, without the need to over-build with heavy structures because of unknown in-flight forces at play.
Wing flutter, or severe vibration, for example, has traditionally been a flight condition that airplane designers wanted to avoid by a large margin because it could signal imminent structural failure.
“Normally we would never fly [aircraft] this close to the flutter boundary. But now, suppose we can; suppose we better understand this. At what point do we start trusting our control system; do we start trusting our sensors?” Chin says.
The “Aha!” moment
Ryu didn’t set out to create a material that could help revolutionize airplane design.
While working toward his 2014 doctorate, he experimented with a commercial polymer that gives off electric current when exposed to light — a mechano-optoelectronic, or MO, material. The polymer could coat the surface of an airplane wing to help detect cracks and corrosion.
Hook the polymer up to an electrode and shine a blue light on it, and cracks can be detected at points where the voltage given off by the polymer increases. Expose the coating to infrared light, and voltage increases could point to spots of corrosion.
But while the coating might be helpful to someone inspecting an airplane on the ground, Ryu was aiming for a material with structural health monitoring capabilities — a material that could help detect potential problems in real time, during a flight. He needed an independent light source for the MO coating to work while a plane was in the air. Then Ryu found his answer. About seven years ago, he attended a SPIE conference, organized for optics and photonics researchers, and learned about preliminary research into mechano-luminescent, or ML materials, which would glow when subjected to vibration or strain. By 2015, Ryu had combined the MO and ML materials to form his MLO composite.
High hurdle: commercialization
Ryu needs to make sure that MLO can be added to the manufacturing process with simple modifications. MLO composite material can be made in a thin film, about 0.5-millimeter thick, and Ryu wants to make it even thinner, which would make it more practical for airplane designers to incorporate. To embed the MLO into a carbon-fiber airplane wing, the builders of the wing could lay the film on a carbon fiber membrane impregnated with resin. From there, the process would be conventional: The carbon composite with the embedded MLO composite would be laid inside a mold and allowed to harden. The wing builders could make the wings with the MLO composite on the inside of the wing, protected from the elements. Multiple layers of the film could provide more information on the structural integrity of the wing — monitoring for both exterior damage and interior strain, for example — although the cost would probably limit the number of MLO layers to two, Ryu says.
Another challenge would be figuring out the best methods for collecting and interpreting the electric current generated by the MLO material.
Electrodes picking up current from the MLO material could be located in a grid throughout the structures monitored on the airplane, and they would pinpoint where damage or strain was occurring on the airplane within the areas of that grid. Airplane designers would need to know how many electrodes would be required over a given area and what levels of voltage would signify strain that was cause for worry. For the wing tests at Armstrong, Ryu plans to build three wing segments. The first segment to be tested will have electrodes at just a few points on the wing to pick up electric current from the MLO composite as the composite senses vibration. The electrodes will be attached to wires carrying the current back to a digital multimeter. The second wing will have more electrodes, and the third wing will have an extensive network of electrodes, similar to how the wing might be designed for a real-world aircraft with structural health monitoring, though Ryu says he hasn’t yet decided how many.
An increase in the structural strain would increase the voltage picked up by the electrodes. The same electrodes would harvest electric current generated by the material, and the current could be stored or put to work for other functions on the aircraft.
Today, structural health monitoring, or SHM, is typically limited to experimental or research aircraft. Accelerometers or strain gauges attached to the wing or other structures do the monitoring. In addition to being lighter than the sensors required with vibration-based SHM, MLO doesn’t need an external energy source. Another advantage of the MLO composite is that it can be embedded inside structures or on their exterior surfaces, and it can detect strain or damage over a spatial area, not just at points where strain gauges are located, for example. Unlike with other materials that generate an electric current only from a limited range of vibration or other mechanical energy, MLO composites generate current from any level of mechanical energy, Ryu says.
Development challenges
One challenge Ryu has faced as he improves the MLO composite has been understanding how the properties of the material change when it is under strain or vibration. For example, the wavelength of the light, or color, and the intensity of the light might tell two different things about the type of damage or strain that the material is sensing. And formulating the material to glow a certain color could boost the levels of voltage generated by the material.
Ryu plans to research how to boost the levels of electrical power generated by the MLO material following this year’s NASA studies.
The future
After the wing testing at Armstrong, Chin says he would like to see how the MLO material performs in further ground testing under variable and extreme temperature and simulated weather conditions. If the material proves itself in the environmental testing, the next step would be flight testing.
As designers push aircraft structural and performance limits to produce greater fuel efficiency, sensors that can tell pilots or flight control computers about the structural health of an in-flight aircraft will play a more important role, Chin says. They could also help minimize inspections or develop new control laws for aircraft. Computers paired with SHM sensors could identify and avoid the type of severe wing flutter that could cause airplane wings or tail structures to explode into pieces during flight, for example. By incorporating SHM into their aircraft, designers wouldn’t have to overbuild the wing structure to stiffen it, which adds weight or bulk to the airplane.
Now, researchers are studying how to incorporate more flexible structures into aircraft, along with creative ways of controlling or suppressing flutter, to make lighter, more efficient aircraft designs possible, Chin says.
A sensor like the MLO composite would have a lot of advantages, because it could be integrated into an airplane’s structure, be self-powered, provide information not available from other sensors and save weight, Chin says. But first, such a sensor will have to prove its reliability.

About Keith Button
Keith has written for C4ISR Journal and Hedge Fund Alert, where he broke news of the 2007 Bear Stearns hedge fund blowup that kicked off the global credit crisis. He is based in New York.
Related Posts
Stay Up to Date
Submit your email address to receive the latest industry and Aerospace America news.
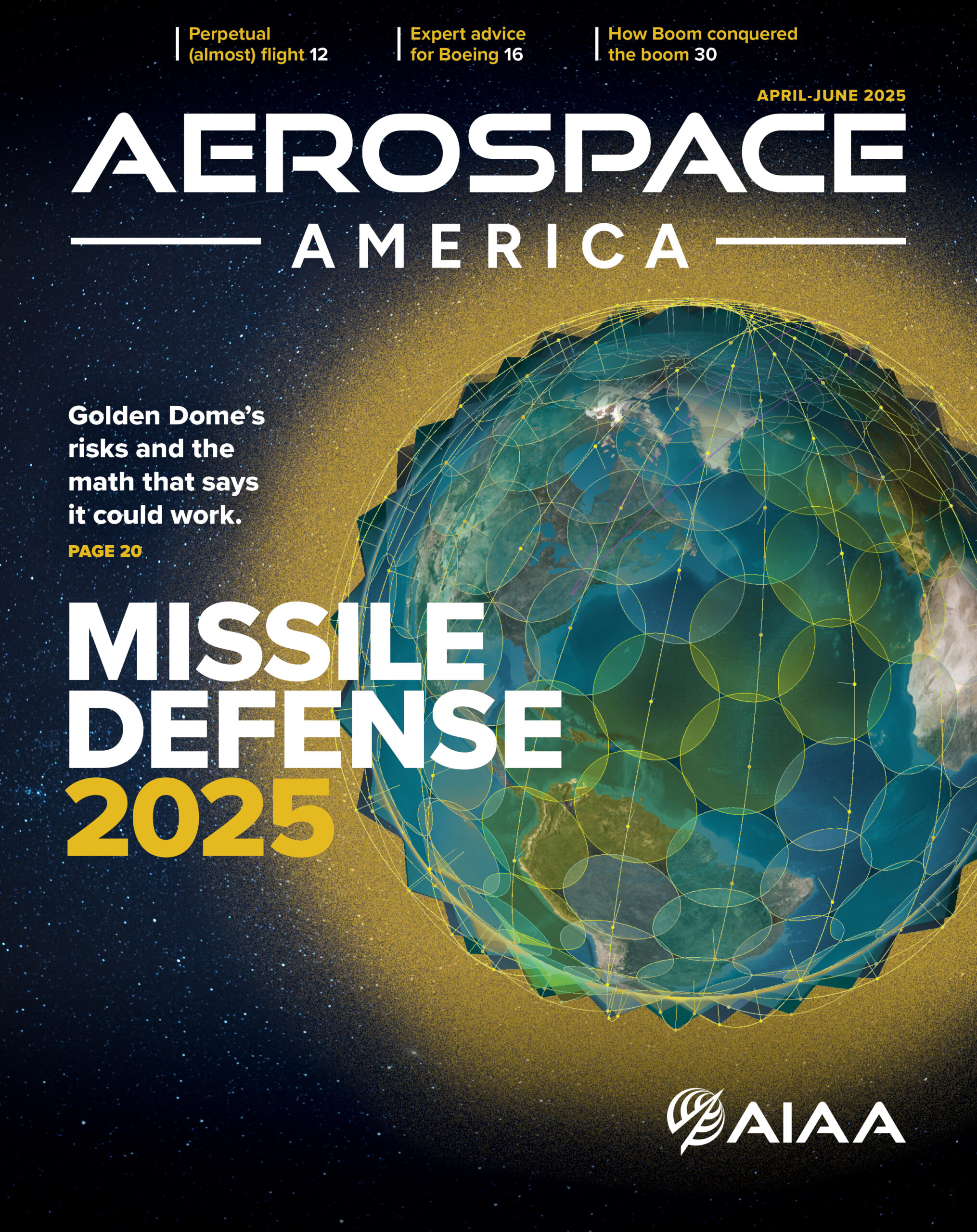
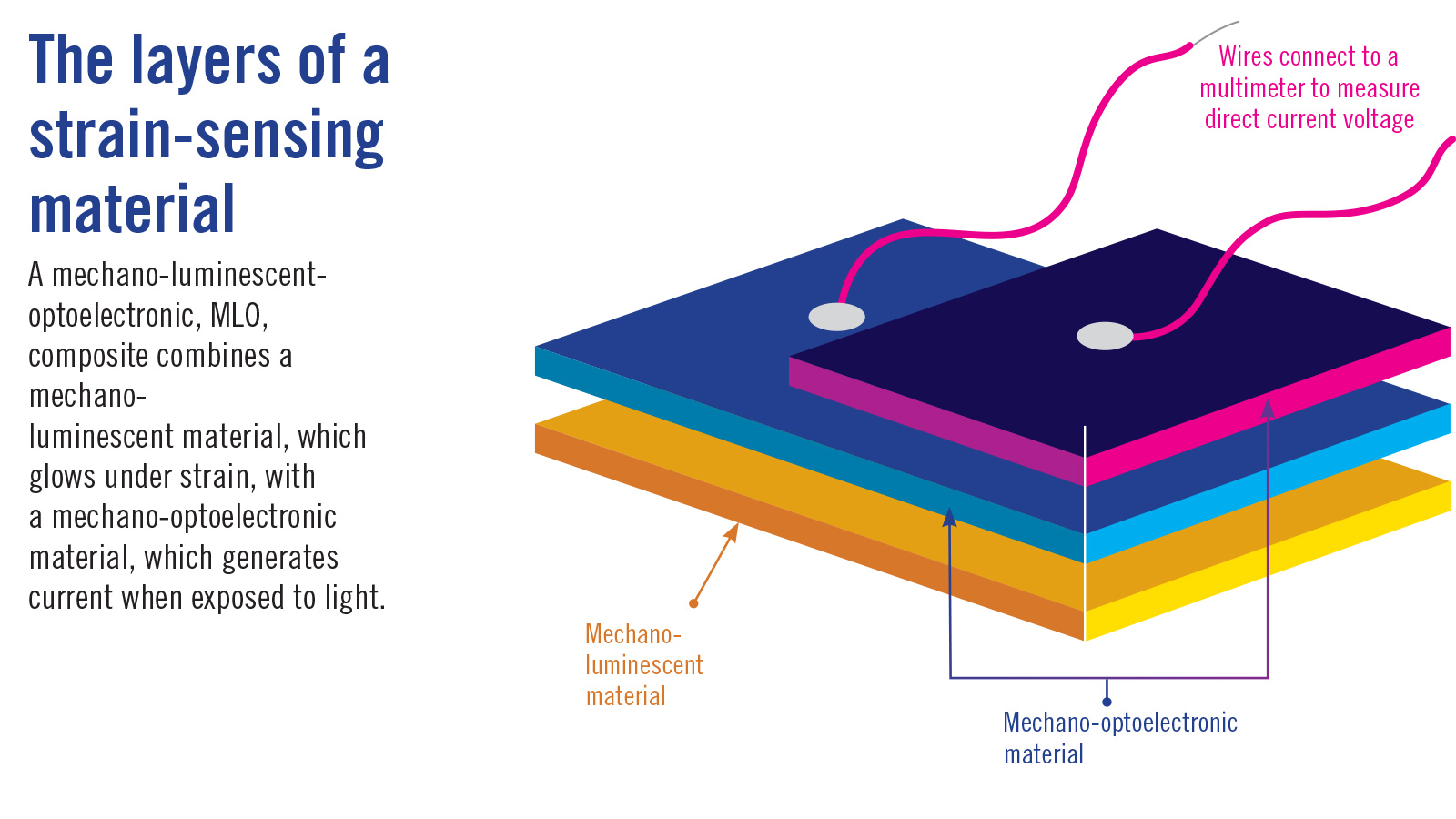
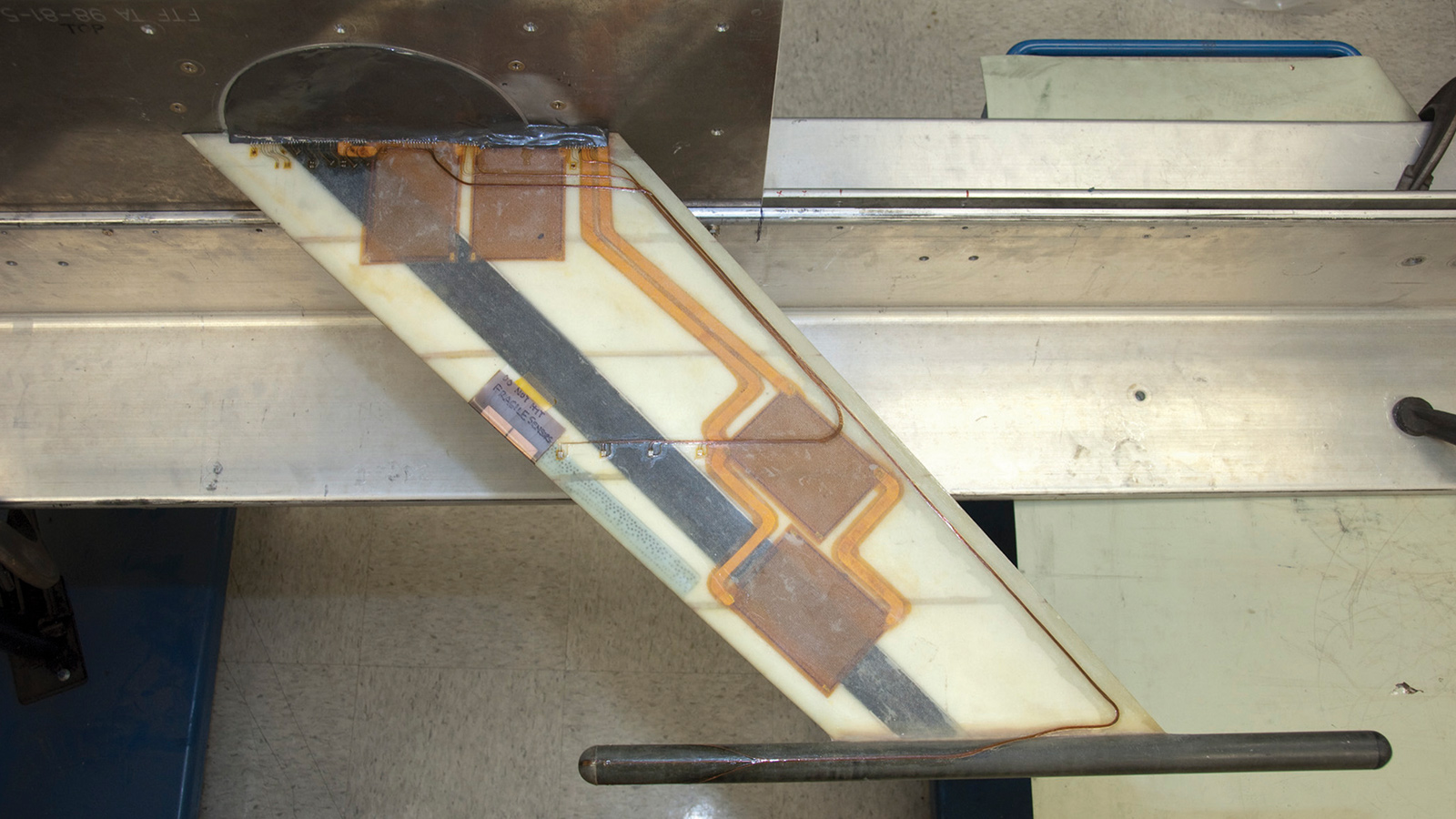
