Stay Up to Date
Submit your email address to receive the latest industry and Aerospace America news.
The air transportation industry wants to achieve net-zero carbon emissions, but its strategy does not include emissionless aircraft. While no one can guarantee the feasibility of such aircraft, especially for long-range flight, NASA’s Dennis M. Bushnell says untapped aerodynamic innovations show tremendous potential toward that goal.
DENNIS M. BUSHNELL is the chief scientist at NASA’s Langley Research Center in Hampton, Virginia
The air travel industry’s current efforts to make long-range air travel kinder to the climate are laudable, but they have an Achilles’ heel: The ideas broached so far would not eliminate aircraft emissions. Today’s turbofan jet engines can burn any of a growing list of approved sustainable aviation fuels made from non-fossil fuel feedstocks. The combustion products from burning these sustainable fuels, however, are similar to when the engines burn conventional fuel. The climate benefit comes from recycling carbon, but today that is done at nowhere near 100%. Next-generation engines might burn hydrogen or operate as hybrids with electricity generated by hydrogen fuel cells, but those aircraft won’t be emissionless either. When they ingest air for combustion, the exhaust will consist of nitrogen oxides and water vapor, which when deposited above the tropopause can form cirrus clouds that block infrared energy emitted from the surface and produce an even larger warming impact than carbon dioxide.
Maximizing air transportation’s efforts to reduce climate forcing will require introducing emissionless aircraft capable of carrying significant numbers of passengers at increasing ranges. Such aircraft represent the frontier of what can be responsibly imagined, but we must stretch our thinking into this realm. To use a pugil analogy, the time has come to take the gloves off in our field to address climate change.
Achieving emissionless flight at ever-increasing ranges will require innovations in aerodynamics, materials and battery electrics to include the improvements in energy density promised by lithium-air, so called because such designs ingest air from the atmosphere. While not ready yet, li-air batteries would be dramatically lighter than today’s lithium-ion versions and, in one estimate, could store seven times more electricity. In the run-up to li-air, we are seeing the development of metal-air and solid-state batteries with greater energy density than lithium-ion.
It’s conceivable that the flight range of a given battery capability could be doubled by employing frontier aerodynamics to dramatically increase lift-to-drag ratio. Work at Northrop in the 1950s by Swiss aerodynamicist Werner Pfenninger, and later by researchers at Virginia Tech, resulted in strut and truss-braced configurations that would at least double the lift-to-drag values but have yet to be implemented for air transport. In the next generation, new aerodynamic configurations, perhaps including strut and truss-braced approaches, could be adopted to vastly increase the lift-to-drag ratio for long-range passenger aircraft, whether those aircraft are powered by sustainable aviation fuels, hydrogen, hybrids or batteries. Doing so would further reduce carbon footprints and increase the range of those aircraft while research proceeds toward the stretch goal of long-range emissionless aircraft.
Here are the most promising aerodynamic and materials innovations to incorporate:
With few exceptions, today’s passenger aircraft do not yet seriously employ laminar flow control to reduce the approximately half drag factor that comes from surface friction. A small passenger aircraft, the Celera 500L, has incorporated an initial laminar flow fuselage for drag reduction. For swept wings, laminar flow control requires suction, which adds additional weight, complexity and cost. Employing externally truss-braced wings, on the other hand, enables the wing to be thinned and its sweep angle reduced so that natural, or pressure gradient, laminar flow control can be employed. The wing truss also provides external structural support to reduce wing weight and significant increases in the wingspan, with outboard wing hinges available to accommodate the parking boxes at airport gates. This increased wingspan much reduces the 35% of aircraft drag that is due to the wing tip vortex formation, termed drag due to lift, which rotates part of the wing lift into the drag direction. Increasing span reduces the portion of the wing affected by the tip vortex.
Also, small longitudinal surface grooves, termed riblets, could be incorporated to reduce the friction drag of surfaces with turbulent flow by up to 10%.
Then there is the very promising ongoing research on plasma turbulent drag reduction approaches, with some 65% reduction in turbulent friction drag achieved thus far, a veritable aerodynamic revolution. Also, locating engines at the rear and utilizing thrust vectoring for control, rather than mechanical elevators or rudders, would obviate the weight and drag of the empennage.
In addition, industry designers could take advantage of trends in weight reduction. There is an ongoing revolution at MIT, NASA and elsewhere toward lighter-weight materials. These include nanocomposites and nano-printed metals, the latter producing superb material microstructures. Advances also are being made toward making the aircraft’s structure double as a battery. As for landing gear, their weight could be reduced by incorporating drag parachutes into operations, alleviating the need for heavy brakes. These parachutes could be deployed in the event of a refused takeoff. Automatic gentle landings would permit the gear to be of a lighter construction. To make truss-braced wings as light as possible, there has been initial consideration in academia of using an inflatable inner wing section. Truss-braced wings already reduce wing weight, due to the external structural support. Utilizing thrust vectoring in lieu of an empennage also would save weight.
The following describes a transport aircraft configuration that would nominally double lift-to-drag ratio by incorporating these innovations:
- A truss-braced wing with doubled span, reduced sweep and thickness, enabling a large drag-due-to-lift reduction and large area of natural laminar flow, and therefore low friction drag.
- Engines or motors moved to the rear. Thrust vectoring would provide control, eliminating the weight and drag of the empennage.
- Fuselage boundary layer engine intake. This design element would increase propulsion efficiency as is well known. It could be configured to produce favorable aerodynamic and propulsion interaction, reducing the effective fuselage drag.
- The fuselage for a small transport would be configured for natural laminar flow, as a current effort has demonstrated. A large transport would employ suction boundary layer relaminarization downstream of the forward passenger door, plasma turbulent drag reduction on surfaces with turbulent flow and digital view screens for the pilots and passengers instead of windows, along with some wall suction for laminar flow control on the fuselage. If the aircraft is powered by hydrogen and that hydrogen is stored cryogenically, cooling of the aircraft wall would be employed to laminarize the fuselage boundary layer.
Preliminary evidence suggests that li-air batteries combined with these approaches could provide fully emissionless ranges approaching 10,000 kilometers. NASA, academia and industry should explore this promising frontier, developing passenger aircraft that are battery-electric with increasing range as advanced batteries and low-drag aerodynamic approaches are deployed. Nearer term, emissionless passenger aircraft would have sufficient range to subsume some half of aircraft travel.
Related Posts
Stay Up to Date
Submit your email address to receive the latest industry and Aerospace America news.
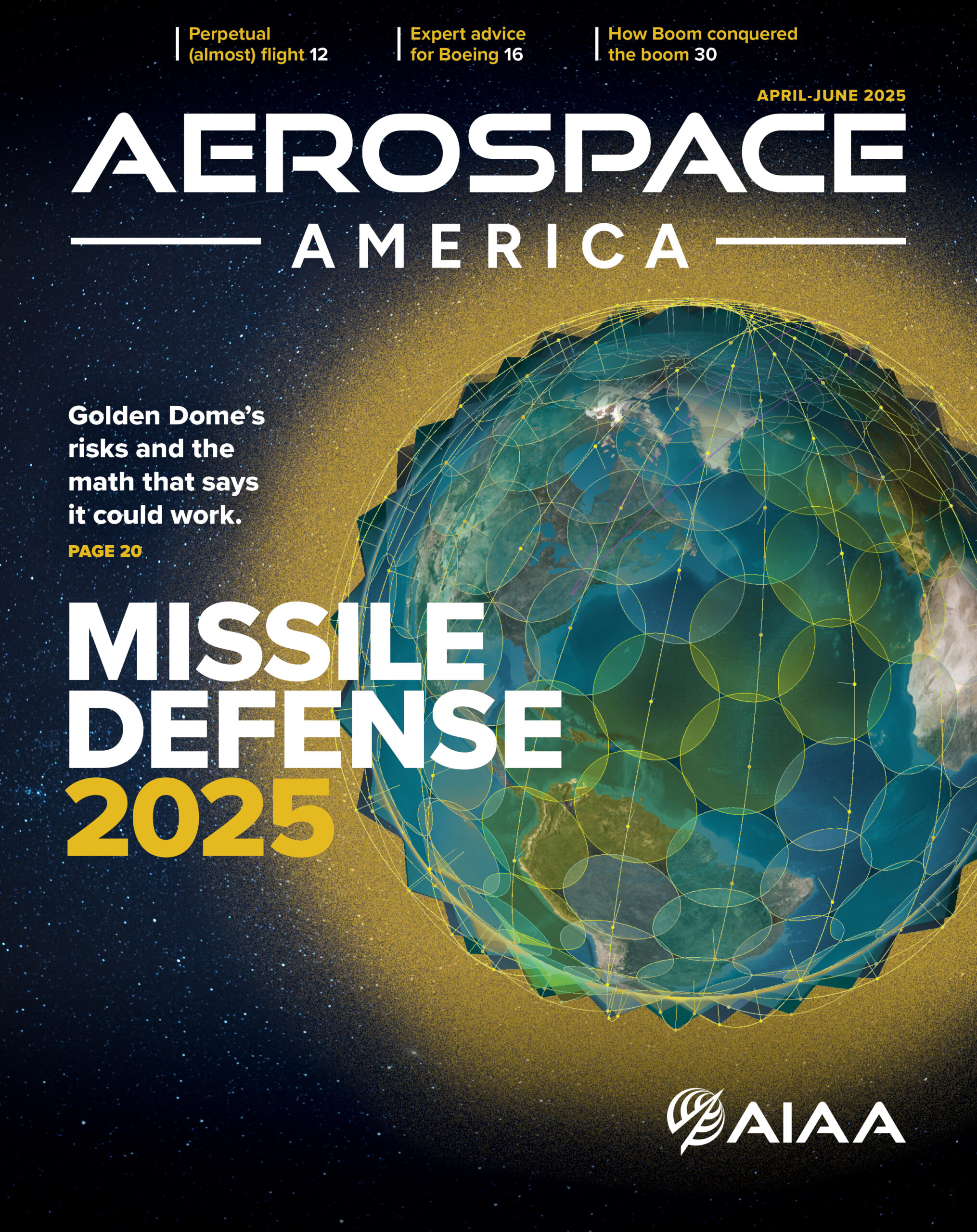